Using Life to Save Lives: Biotechnology and Healthcare
By Scott Reddiex
Editor-in-Chief, Science Victoria
The COVID-19 pandemic made many terms commonplace: mRNA vaccines, antibodies, antigens (in rapid antigen tests (RATs)), and antivirals would now be familiar to most.
Each of these was produced en masse to ensure that everyone had access to a RAT, a vaccine dose, or intensive therapies if their infection was severe. But how exactly are they made?
The answer, broadly, is biotechnology: the harnessing of biological organisms and systems to produce a substance or perform a task. Most often, these organisms are bacteria, fungi, or plants. While postgraduate students and research assistants do not fall under this definition of ‘task-performing biological organisms’, animals can also be utilised in biotechnology – for example, growing human organs in pigs.
There are many and varied applications of biotechnology in modern medicine, with several examples from the COVID-19 pandemic.
A Recent History of Biotechnology
As you will have noted from the above definition, biotechnology is nothing new. Humans have been using yeasts for baking and brewing for thousands of years, and many societies have utilised various plants in primitive remedies for injury and illness. Even the development of the lemon through the hybridisation of two different citrus species is an example of early biotechnology – life didn’t give us lemons; humans brought them into existence.
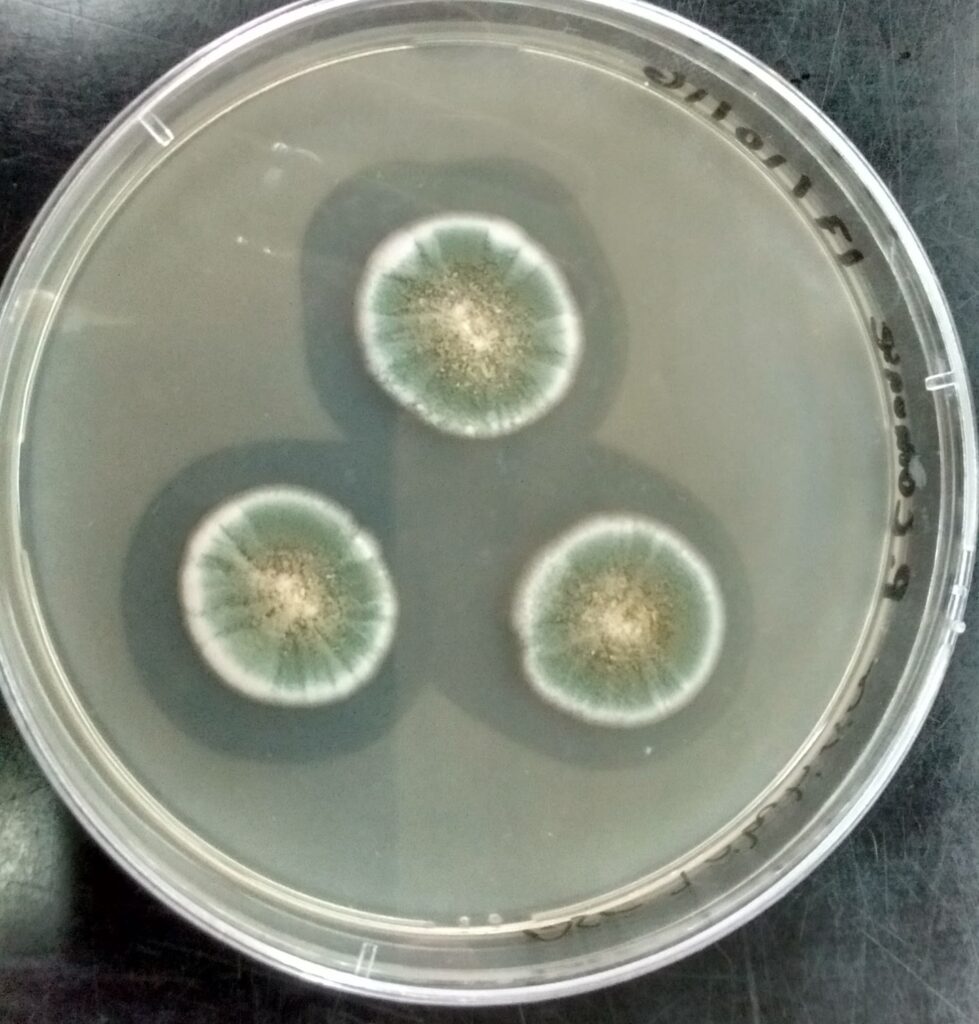
The journey of penicillin, from observation to understanding and harnessing the organism that makes it, is a clear example of how biotechnology can transform natural processes into practical medical applications.
From the late 1800s, several scientists had observed that some moulds were able to inhibit the growth of bacteria, but wasn’t until 1928 that Sir Alexander Fleming made his chance finding that Penicillium notatum could inhibit the growth of the bacterium Staphylococcus aureus.1,2
Fleming carefully studied the mould and its effects, but was unable to isolate and purify the molecule responsible for the observation, leaving penicillin unsuitable for use as a therapeutic drug. It wasn’t until the work of Sir Howard Florey and others in the 1940s that the molecule was identified, the production was improved and scaled up, and the medical applications of the first commercial antibiotic were realised.1,2
Further research has allowed for massively increased yields in shorter time, as well as different forms of the antibiotic that have increased bioavailability (i.e., can be better used by the body), or are able to overcome bacterial resistance.
Research across multiple fields, as well as advancements in engineering, have not only allowed us to better understand how organisms have their observed effects, but also to harness, modify, and exponentially scale up those effects. While these processes are now commonplace in labs, they are rarely on display like they were during the COVID-19 pandemic.
mRNA and other vaccines
Discussion of COVID-19 vaccines dominated the news of 2020 and 2021. As researchers and clinicians pivoted into COVID-19 research, and public and private money was thrown at the task, multiple vaccine candidates began to emerge.

At this point in time, we already had centuries of knowledge relating to vaccination, and how humans generate a protective immune response. At its core is the principle of showing the right parts of an invader to the right parts of the immune system so that a specific and protective memory is acquired.
Importantly, we don’t want the patient to contract the illness in the process, and therefore need to carefully select what will be included in a vaccine. We also need to remember that not every part of the invader is able to generate a helpful immune response.
Vaccines typically address these requirements by combining key things in each dose: something that looks like the right part of the invader, and something to get it to the right place in the body. If all goes well, the next time we see that exact part of the invader, we’ll be prepared.
AstraZeneca, Moderna, and Pfizer
In the case of COVID-19 (the disease caused by the virus, SARS-CoV-2), the different vaccines that reached the arms of Australians were AstraZeneca’s Vaxzevria, Moderna’s Spikevax, and Pfizer-BioNTech’s Comirnaty.3,4,5
AstraZeneca’s vaccine used an adenovirus vector – the inactive shell from a different kind of virus – to get the payload into cells. In this case, that payload was a strand of DNA, which served as instructions for cells to make copies of the SARS-CoV-2 spike protein – the ‘right part of the invader’.3
Pfizer and Moderna’s vaccines are mRNA vaccines. In this case, lipid nanoparticles act as transporters to deliver mRNA instructions directly into the cells, without the need for a viral vector.4,5
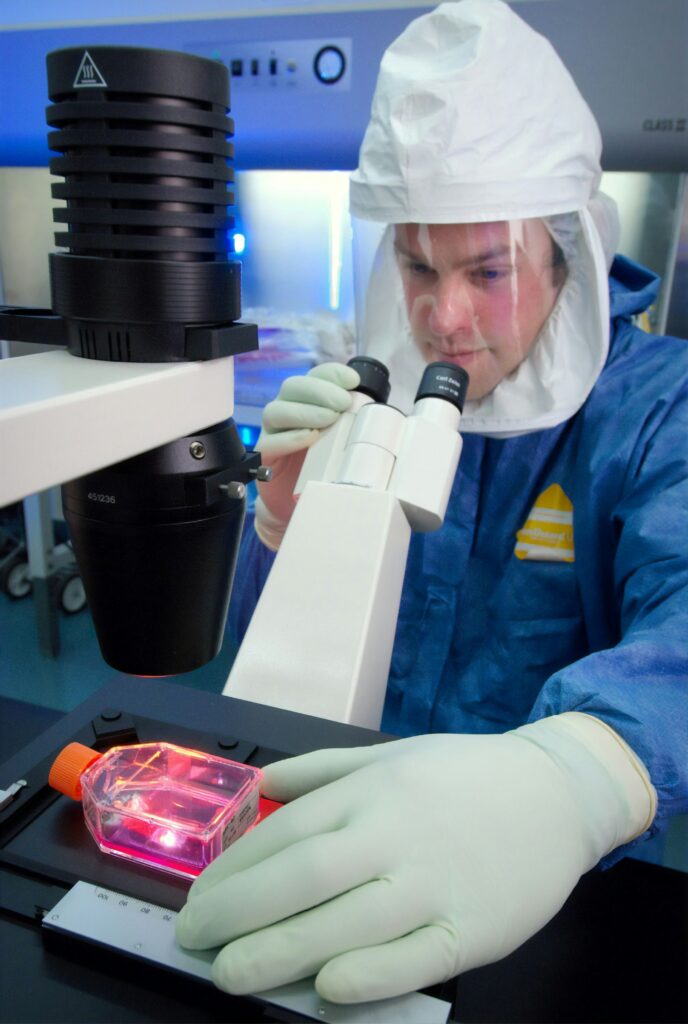
Both the mRNA and the DNA vaccines provide instructions for the recipient cells to make the virus’s spike protein, for the immune system to learn to recognise it. DNA is read to make mRNA, which is in turn read to make a protein, meaning that an mRNA vaccine has fewer steps before the goal is achieved.
Understanding what each of these vaccines contains makes it easier to see how biotechnology is involved in their production. For AstraZeneca, the DNA that encodes the adenovirus is modified to both make sure that it contains the DNA sequence of the SARS-CoV-2 spike protein, while also ensuring it can’t replicate on its own – it is a virus itself, after all.6
This modified virus is then grown inside human cell lines – vats of ‘immortalised’ cells that reproduce like tumour cells – before being harvested, purified, and included in the vaccine formulation.6
In contrast, the production of mRNA vaccines doesn’t involve cells or animal-derived raw materials. Instead, a DNA template is combined with the other ingredients and catalysts required for the mRNA to be produced. The process works using the same principles as mRNA production inside our cells, however it uses machinery derived from bacteriophages (viruses that infect bacteria).7
The mRNA production process is significantly quicker and produces milligrams of mRNA for every millilitre of reaction volume, which is a sizable yield. The mRNA is then purified, and encapsulated in lipid nanoparticles, ready for vaccine formulation.7
While these vaccines are great at reducing the chance and severity of infection, most of us by this stage have had COVID-19. Apart from the obvious and unpleasant symptoms, the main method for confirming a bout of COVID-19 is the use of a RAT.
Rapid Antigen Tests (RATs)
A “rapid antigen test” is exactly that – a test that rapidly determines if a given antigen is present in a sample. An antigen is anything that is recognised by the immune system – usually part of a protein.
While a RAT might seem more on the ‘tech’ side, it relies on a ‘bio’ component to work: antibodies. Antibodies are proteins produced by specific immune cells, with each cell producing unique clones with specificity for a single antigen.
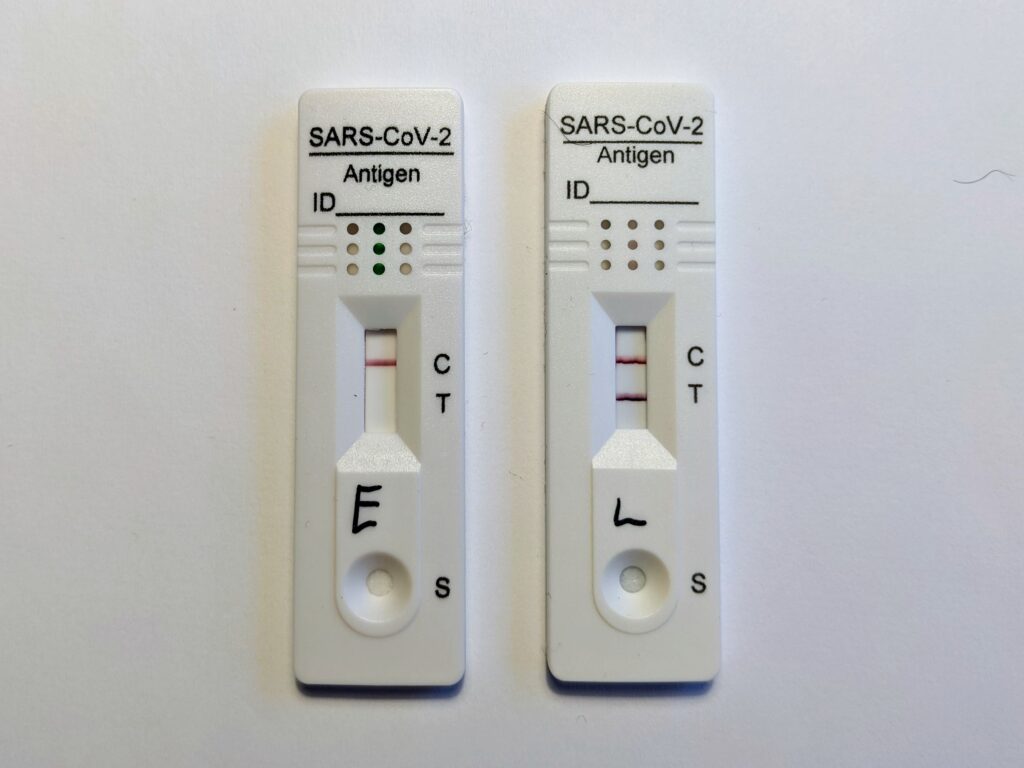
It is this specificity of an antibody for an antigen that underpins how a RAT works, and is the same principle that a home pregnancy test uses (the antigen in those is a pregnancy-related hormone). The process occurs in stages:8,9
- Viral proteins (antigens) present in nasal fluid are caught on the swab, and then rinsed into the provided buffer solution. The buffer solution releases any SARS-CoV-2 proteins from cells and mucus, optimises the pH, and forms the carrier solution for the test.
- The sample solution is dripped onto, and begins to flow across, the test strip. As it passes through different sections of the strip, the solution encounters various components essential for detecting viral proteins.
- The solution first passes through a section called the conjugate pad, which contains antibodies specific to SARS-CoV-2 antigens. These antibodies are labelled with small particles, such as gold, latex, carbon, or silver, so that a colour change can occur (the lines that appear).
- If SARS-CoV-2 antigens are present, the antibodies bind to the antigens, forming a protein-antibody complex that continues flowing along the strip. If no antigens are present, the labelled antibodies flow alone.
- At the “test” line, immobilised antibodies that bind specifically to the protein-antibody complex are present. If SARS-CoV-2 antigens are present, the complex binds to these antibodies, causing a visible coloured line to appear.
- Finally, at the control line, a different set of immobilised antibodies bind to any unbound labelled antibodies. This ensures the test is functioning properly.
Producing the antibodies required for the RAT is the biotechnological part of the process. First, animals (like mice, rats, and goats) are injected with an antigen, like a protein from the surface of SARS-CoV-2. Over the next few weeks, the animal’s immune system produces antibodies specific to that antigen.10
The animals producing the “best” antibodies are identified, and their antibody-producing cells are collected. These cells are then fused with cells that can grow indefinitely – derived from cancerous antibody-producing cells. The result is a “hybridoma”, a type of cell that can both produce antibodies and reproduce continuously.10
These hybridomas are grown in bulk, with each one producing large quantities of the specific antibodies required for the RAT. The antibodies are then harvested, purified, and impregnated into the test strip at the appropriate section, ready for your next COVID-19 test.
However, hybridomas aren’t the only way to produce antibodies used for treatments.
Antibody therapies
For COVID-19 patients who are very sick, antibody therapies are sometimes used to treat their infections.11,12
The primary function of these therapies is to neutralise the virus. When each virus particle is bound to a (relatively) large antibody, it is physically blocked from attaching to and infecting our cells. In other words, if we think of the virus as a key, and the patient’s cells as a lock, then the antibody therapy is like supergluing a bell to the tip of the key – the key won’t be able to fit in the lock, and it’s going to make a lot of noise while trying.
The secondary function of these therapies is to make the virus visible to different parts of the immune system. That’s the bell in the above analogy, signalling to other immune cells and defence molecules that this is a target to attack.
The antibodies used for this therapy have two sources: people, and immortalised cell lines. If someone has generated an immune response to COVID-19 (either through infection or vaccination), their blood will contain antibodies specific to parts of the SARS-CoV-2 virus. This means that blood can be donated, and their antibody-containing sera collected and then used to treat a patient – similar to how a blood transfusion works.11
Larger-scale and more specific antibody therapies are produced similarly to the antibodies used in RATs, but with some key differences. First, candidate antibodies are selected, their structure studied and optimised, and a gene encoding that protein created. Next, that gene is introduced into an immortalised cell line (such as Chinese Hamster Ovary (CHO) cells), and grown in large cultures.13 The output is a single type of highly specific antibody that can then be purified and administered to patients.
Biotechnology is a fundamental part of modern medicine
Processes and techniques that fall under the definition of ‘biotechnology’ are central to how we responded to COVID-19, from test kits, to vaccines, and therapies.
These methods aren’t restricted to COVID-19, either. You’ve possibly seen RATs that test for multiple infections, or used a home pregnancy test. You’ve hopefully kept up-to-date with your vaccines, and you hopefully won’t be needing antibody therapy for illnesses like rabies, Ebola, various cancers, some autoimmune diseases, or many others.
As biotechnological processes continue to improve, so too will our ability to rapidly and effectively respond to a wide range of diseases.
References:
- Macfarlane, G. (1985). Alexander Fleming: the man and the myth. Oxford University Press.
- Judson, H.F. (1987). The Search for Solutions. Holt Rhinehart & Wintson.
- Voysey, M., et al. (2020). Safety and efficacy of the ChAdOx1 nCoV-19 vaccine (AZD1222) against SARS-CoV-2: an interim analysis of four randomised controlled trials in Brazil, South Africa, and the UK. The Lancet, 397(10269). doi.org/10.1016/s0140-6736(20)32661-1
- Vogel, A. B., et al. (2021). BNT162b vaccines protect rhesus macaques from SARS-CoV-2. Nature, 592(7853), 283–289. doi.org/10.1038/s41586-021-03275-y
- Jackson, L. A., et al. (2020). An mRNA Vaccine against SARS-CoV-2 — Preliminary Report. New England Journal of Medicine, 383(20). doi.org/10.1056/nejmoa2022483
- Kovesdi, I., & Hedley, S. J. (2010). Adenoviral Producer Cells. Viruses, 2(8), 1681–1703. doi.org/10.3390/v2081681
- Rosa, S. S., et al. (2021). mRNA Vaccines manufacturing: Challenges and Bottlenecks. Vaccine, 39(16). www.ncbi.nlm.nih.gov/pmc/articles/PMC7987532/
- Shirazi, S., et al. (2021). Testing for COVID-19 in dental offices. The Journal of the American Dental Association, 152(7), 514-525.e8. doi.org/10.1016/j.adaj.2021.04.019.
- S. Pavia, C., & M. Plummer, M. (2021). The evolution of rapid antigen detection systems and their application for COVID-19 and other serious respiratory infectious diseases. Journal of Microbiology, Immunology and Infection, 54(5), 776–786. doi.org/10.1016/j.jmii.2021.06.003
- Hybridoma technology. Wikipedia; Wikimedia Foundation. en.wikipedia.org/wiki/Hybridoma_technology
- Hammarström, L., et al. (2021). Antibody therapy for COVID-19. Current Opinion in Allergy & Clinical Immunology, 21(6), 553–558. pmc.ncbi.nlm.nih.gov/articles/PMC8577309/
- San Filippo, S., et al. (2022). Comparative efficacy of early Covid-19 monoclonal antibody therapies: a retrospective analysis. Open Forum Infectious Diseases. doi.org/10.1093/ofid/ofac080
- Dhara, V. G., et al. (2018). Recombinant Antibody Production in CHO and NS0 Cells: Differences and Similarities. BioDrugs, 32(6), 571–584. doi.org/10.1007/s40259-018-0319-9